Railsback, J., & Corey B. (2003). Building Trust With Schools and Diverse Families.
Abstract
Capable of generating plasmonic and other effects, aureate nanostructures can offer a variety of diagnostic and therapy functionalities for biomedical applications, but conventional chemically-synthesized Au nanomaterials cannot e'er match stringent requirements for toxicity levels and surface conditioning. Light amplification by stimulated emission of radiation-synthesized Au nanoparticles (AuNP) present a feasible alternative to chemical counterparts and tin can offer exceptional purity (no trace of contaminants) and unusual surface chemistry making possible straight conjugation with biocompatible polymers (dextran, polyethylene glycol). This work presents the outset pharmacokinetics, biodistribution and safety written report of laser-ablated dextran-coated AuNP (AuNPd) nether intravenous administration in small animal model. Our data evidence that AuNPd are quickly eliminated from the claret apportionment and accumulated preferentially in liver and spleen, without inducing liver or kidney toxicity, as confirmed by the plasmatic ALAT and ASAT activities, and creatininemia values. Despite sure residual accumulation in tissues, we did not detect any sign of histological damage or inflammation in tissues, while IL-half-dozen level confirmed the absence of whatsoever chronic inflammation. The safety of AuNPd was confirmed by healthy behavior of animals and the absence of astute and chronic toxicities in liver, spleen and kidneys. Our results demonstrate that laser-synthesized AuNP are safe for biological systems, which promises their successful biomedical applications.
Introduction
Gilded (Au) is one of very important elements of the periodic table, known by both luxury qualities and unique physical, physico-chemical and chemical characteristics. The reduction of size of aureate down to the nanoscale leads to novel astonishing properties such as prominent catalytic1,2 and electrocatalytic3,four responses, a variety of plasmonic effects associated with optical excitation of collective complimentary electron oscillations (surface plasmons), including strong resonant extinction5, drastic field enhancement6, optical manipulations across the diffraction limit7, ultrasensitive biosensingeight, etc. On the other hand, gold is highly biocompatiblenine,x, which promises successful biomedical applications of Au nanoparticles (AuNP) profiting from plasmonic or other effects11. Oncology looks as one of most promising application areas, equally after an appropriate functionalization AuNP tin be targeted to the tumor (actively or passively), accumulated in cancer cells and so be used either as contrast agents in cancer imaging or sensitizers of unlike local therapies under external stimuli. As an example, extremely efficient optical assimilation, with typically ~10v-fold higher cross department than absorbing dyes, has been used in a number of diagnostic and therapeutic modalities, including light induced hyperthermia-based therapy12,13,14, confocal reflectance microscopyfifteen, photoacoustic tomography16, optical coherence tomography17 imaging modalities. For these optical modalities, Au nanostructures should have their absorption/scattering feature within the tissue transparency window between about 700 and 1000 nm, which tin be satisfied past employing designed plasmonic structures (SiO2-Au or Si-Au core-shells, Au nanorods). As another prominent example, relatively small (5–20 nm) AuNP tin serve as dissimilarity agents in X-ray radiations imaging18,19 and as sensitizers of radiation therapiestwenty,21,22.
Such biomedical applications require the pick of minimally toxic Au-based nanomaterials to enable such imaging or therapeutic functionalities. The toxicity of Au nanostructures depends on size and shape (spherical, nanorods, nanostars, etc) characteristics, likewise as surface conditioning and chemistry, including the presence of toxic contaminants, surface charge and stabilization coatings23,24,25,26. Conventional methods for the synthesis of colloidal Au nanostructures (nanoparticles, nanorods, core-shells) are based on colloidal chemistry, including reverse micelles or the reduction of a gold precursor in the presence of capping agents.13,14,xv,27,28,29,30, but these methods cannot provide optimal parameters for biomedical applications. Get-go, these methods typically cause surface contamination by rest by-products, ligands and reducing agents, which can crusade toxicity problems31,32,33. Second, in many cases chemically-synthesized AuNP are positively charged or stabilized by cationic surfactants, which tin provoke their undesirable interactions with negatively charged DNAs34,35,36. Tertiary, the stabilization of chemically-synthesized AuNP and avant-garde structures (nanorods, nanocages, nanostars etc.) typically requires surfactants and protecting ligands that tin can lead to severe toxicity effects. Every bit an instance, the stabilization of nanorods is based on the utilize of cetyl trimethylammonium bromide (CTAB)25,26, which causes unexpectedly high toxicity24,37. The biocompatibility of chemically-synthesized Au nanostructures can be much improved by their coating by polymers such as polyethylene glycol (PEG), dextran, polyvinyl-pyrrolidone (PVP), poly (acrylic acrid) (PAA), and polyvinyl-alcohol (PVA)23,24, simply such coating cannot completely protect from the release of toxic agents later on a long-term exposure of AuNP-polymer complexes in vivo. The evolution of AuNP in biological systems, including efficiency of cellular uptake, biodistribution etc., is finally highly afflicted by a poly peptide corona (layer of proteins that adsorb on the nanoparticles in biological environments), just the limerick of this corona is likewise strongly affected by initial surface conditioning and coatings23,24,25. In general, spherical nanoparticles with the size effectually 15–fifty nm look less toxic, provided they do not comprise toxic contaminants and are properly coated23,24,25,26.
Laser-ablative synthesis was recently introduced as a "green" physical culling to conventional chemical pathways, which offers quite unlike surface workout and chemistry, also equally an exceptional level of fabric purity38,39,xl,41. Such a synthesis profits from natural generation of nanoclusters under ablation of a solid target by laser radiations in liquid ambient42. The nanoclusters then coagulate in the liquid to form colloidal nanoparticle solution. Since this method does not depend on the presence of specific chemic products (e.thou., aureate salts) during the synthesis, it can avoid any contagion of nanoparticle surface, while the use of ultrashort (femtosecond) light amplification by stimulated emission of radiation ablation enables to finely control size and structural characteristics of formed AuNP40,41,43,44,45. Information technology is besides of import that laser-synthetized AuNP exhibit unusual surface chemistry based on the presence of golden oxide states, which conditions O− termination when pH >546. Such a surface chemistry, hardly reproducible with chemical synthesis pathways, makes possible hydrogen bonding interactions of AuNP with a diversity of biocompatible molecules containing OH groups, including oligosaccharides46,47, biopolymers (polyethylene glycol (PEG), dextrans)48, proteins49 and oligonucleotidesfifty. The one-step conjugation with polymers or other molecules (no intermediate group is required) presents an interesting new choice, which could requite some advantages for biological applications. In addition, laser-ablated AuNP (bare or polymer-coated) are typically negatively charged, which promises the absence of toxic effects related to DNA damage.
Ultrapure AuNP prepared past laser ablation could be considered equally new appealing candidate for biomedical applications, but there are but a few data describing their interaction with biological systems. Studying the interaction of laser-synthesized AuNP with rat pancreatic tumour cells, Goldys et al.51, reported their non-toxicity and a size-dependent cellular uptake. Barcikowski et al. showed that the functionalization of light amplification by stimulated emission of radiation-produced AuNP with some peptides52 and polymers53 can improve prison cell uptake. Nosotros recently studied cytotoxicity and cell uptake of three types of Au nanoparticles: bare (ligand-free) AuNP, dextran-coated AuNP and PEG-coated AuNP54. Cell viability profiles demonstrated that AuNP did non induce major inhibition of cell survival until concentrations of 100 µg/mL, indicating a satisfactory low toxic effect, while all type of AuNP demonstrated a good cellular uptake. By examining the interaction of blank, dextran- and PEG-coated AuNP with living cells, for the first time we determined the composition of the poly peptide corona covering the nanoparticles in biological environment. Such a corona appeared after 30 seconds of AuNP exposition and contained a variety of proteins, including apolipoproteins, which are implicated in intracellular ship and crossing of biological barriers, while the presence of immune proteins (complement C3) was lower for polymer-coated AuNP compared to blank ones, suggesting their better ship properties.
This study is conceived as the showtime attempt to appraise the interaction of dextran polymer-coated light amplification by stimulated emission of radiation-synthesized AuNP with biological systems in vivo. Using intravenous administration of AuNPd in mouse model, nosotros examine toxicity, biodistribution and pharmacokinetics of these nanoparticles. Nosotros bear witness that despite certain rest aggregating in organs of reticuloendothelial organisation, light amplification by stimulated emission of radiation-synthesized AuNPd look safe and exercise not provoke any sign of toxic effects.
Results
Synthesis and characterization of dextran-coated Au nanoparticles
For fabrication of AuNP, we used a technique of fs laser ablation in aqueous solutions, which was adult in our previous studies46,47,48. A gilt target was placed on the bottom of a glass vessel filled with an aqueous solution of dextran (i mg/mL) and ablated by radiation from a femtosecond Yb:KGW laser (see details in Methods section), equally shown in Fig. 1A. The ablation process led to the production of colloidal dispersions of AuNP, which was accompanied by a characteristic carmine coloration of solutions. The formed solutions exhibited a characteristic peak around 520 nm in the extinction spectra, which is associated with the excitation of surface plasmons over aureate NP. As nosotros showed in our before studies46,47,48,54, Au nanoparticles prepared by the technique of femtosecond laser ablation in aqueous solutions exhibit exceptionally high stability (they are stable for several years while keeping at room temperature) due to electric repulsion event resulting from strong negative charging of nanoparticles. In our instance, AuNPd were also very stable, as nosotros did not see any sign of agglomeration or precipitation afterward several months of storage in glass vials. According to manual electron microscopy (TEM) images and size distribution analysis (Fig. 1B), particles prepared in dextran solution had the mean size of 21 nm and size dispersion less than 10 nm total-width-at-half maximum. As nosotros earlier showed54, hydrodynamic diameter of such dextran-coated AuNPd is about 46 nm, which is consistent with the germination of Au nanoparticle – dextran complexes.
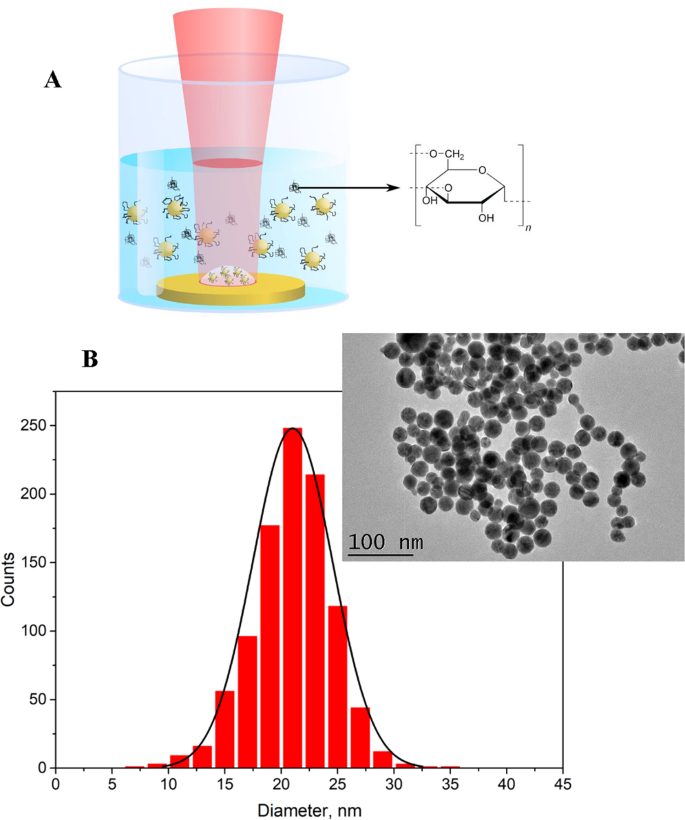
(A) Schematics of laser ablation setup. A laser axle is focused on the surface of the Au target, which is placed in the vessel filled with an aqueous solution of dextran. (B) Typical transmission electron spectroscopy (TEM) image and corresponding size distribution of AuNP synthesized past pulsed light amplification by stimulated emission of radiation ablation in aqueous solution of dextran.
Effects of AuNPd on body weight
Three groups of six mice were administered with a single intravenous dose of one mg/kg of AuNPd and the control group was injected with vehicle alone. To investigate potential toxic effects due to NP, body weight and mice behavior were monitored after AuNPd administration. All animals showed a salubrious appearance and normal activity without lethargy or aloofness later NP administration. During the first 14 days after subcutaneous tumor grafting, as well equally during the fourteen days following AuNPd injection, the body weight of the mice injected with NP slightly increased in a design like to the control mice, suggesting a normal mice growth in the absence of any significant toxic effect (Fig. ii). Iii mice of each group were placed in metabolic cages 24 h before the sacrifice (which occurs 24 h, 7 days or 14 days after AuNP administration), to monitor their food and h2o consumption and to collect urines for farther determination of gilt content. We did not observe any difference in water intake or food consumption between treated and control groups.
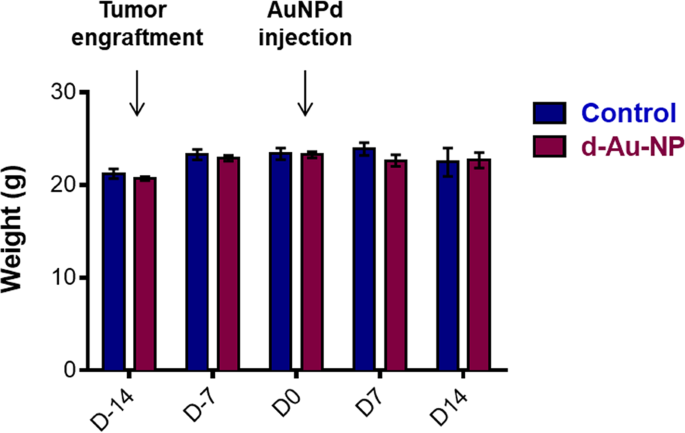
Development of the hateful torso weight of control mice or mice exposed to one mg/kg AuNPd. Mice were weighted twice a week throughout the 28 days of the follow-upward experiment; data represent the mean ± SEM of 6 mice.
Pharmacokinetic assay
To determine pharmacokinetic profile of AuNPd in blood, 7 groups of five athymic nude female person mice were treated with AuNPd at a unique dose of 1 mg/kg by Four injection. Gold concentration was determined by inductively coupled plasma mass spectrometry (ICP-MS) 5 min, 15 min, thirty min, 45 min, 60 min, four h and 24 h after AuNPd injection. To forestall underestimation of gold concentration that could be due to an incomplete mineralization of nanoparticulate gold, solutions were dosed with calibration curves carried out with ionic gold standard solutions and golden nanoparticles standard solutions. No meaning differences were noted whatever the standard solutions used, confirming validity of the measurement (data not shown). AuNPd blood concentration-time bend with a semilogarithmic calibration evidenced a rapid driblet in initial concentration, followed by more than gradual decline fitting into a classical bi-compartmental pharmacokinetic model characterized by a fast initial distribution phase followed past a last elimination phase with slower rate (Fig. three). Mean pharmacokinetic parameters, calculated using bi-compartmental analysis are summarized in Tabular array 1. One 60 minutes subsequently injection, more than than 95% of the injected dose was eliminated from the blood circulation, which is consequent with the low value of T1/2α (one-half-life for the distribution stage). Ti/2α is the time required for the blood level of AuNPd to decrease by 50% of its initial value. Gold was undetectable in blood 24 h post-administration, thus explaining the brusque T1/2β (half-life for elimination) and the loftier clearance value. The Vd parameter that reflects the degree of how a foreign material is distributed in organs and tissues, was well beneath the book of the mice body fluid (2 ml), which meant that AuNPd accumulated in the organs. All together, these information suggest that the amount of AuNPd in the key compartment (blood) declines rapidly due to the transfer of NP to the peripheral compartment (tissues) and/or to AuNPd elimination that occurs simultaneously.
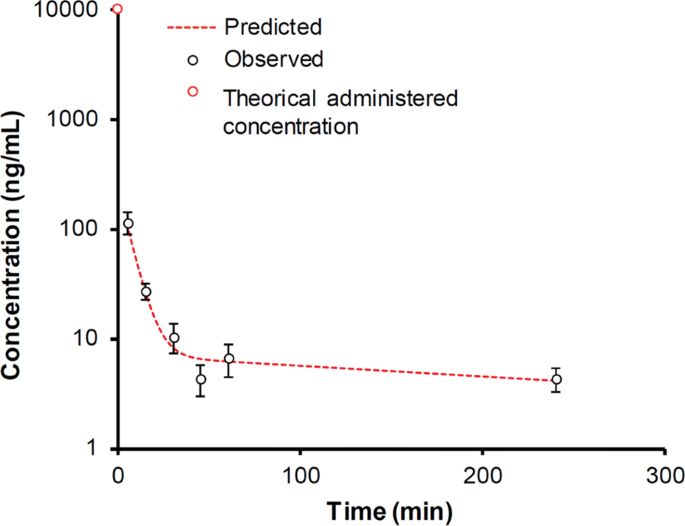
Pharmacokinetic contour of AuNPd (ane mg/kg) at 5, 15, 30, 45, 60 min and iv h after a single intravenous assistants (northward = five, data are mean ± SEM). A signal for 0 infinitesimal corresponds to theoretical administered concentration (ten 000 ng/ml).
Tissue biodistribution of AuNPd
Pharmacokinetic parameters betoken a rapid clearance of AuNPd from the bloodstream. To determine the distribution of AuNPd in tissues, three groups of 6 mice were administered with i mg/kg of AuNPd and sacrificed at different times after injection: 24 h, 7 and xiv days. The control group was injected with vehicle lonely and sacrificed at day 14. Gilt concentration in various tissues, including liver, spleen, kidney, eye, lung and brain, was measured by ICP-MS and expressed as ng/mg organ. Twenty-four hours after injection, spleen and liver were preferential sites for gold accumulation with 8.78 ± four.33 ng/mg and half-dozen.36 ± 3.37 ng/mg (Fig. 4A et 4B), respectively. However, considering size of the organs, AuNPd accumulated mostly in liver, in which Au concentration was more than 35% of the injected dose after 24 h and more than l% after one and two weeks (Table ii). In other organs and in tumor, gold concentrations were much lower with less than 0.fifteen ng/mg of tissue at xiv days. The lowest concentration was found in the brain with less than 0.03 ng/mg. Moreover, no statistical difference was found between gold concentrations at unlike time point in lungs, heart, encephalon and urine. In addition, AuNPd were not cleared by kidney considering less than 0.ane% of injected dose was found in urine (Fig. 4C). All together, our results indicate that AuNPd accumulated mostly in the liver, without obvious subtract for 14 days.
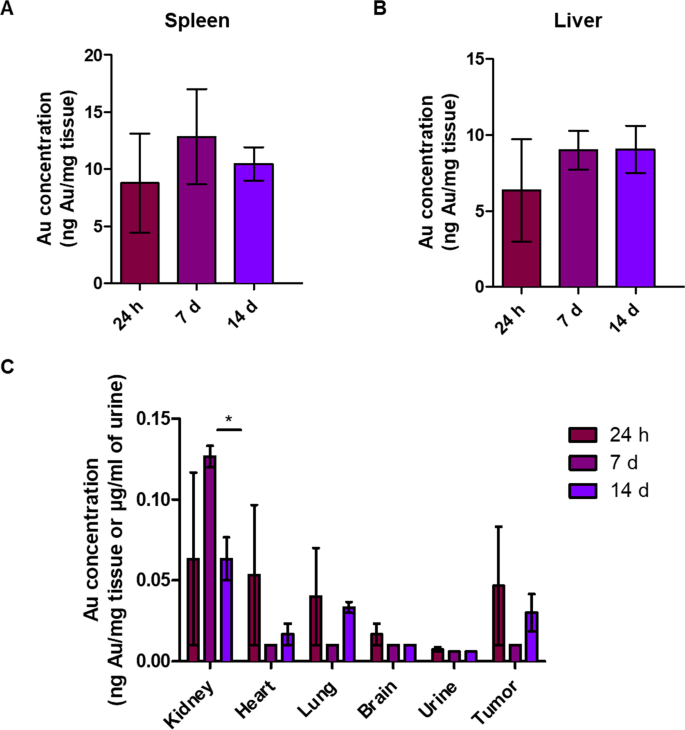
Gold concentration measured past ICP-MS 24 h, seven days and xiv days after a unmarried injection of AuNPd (1 mg/kg). (north = three, data are mean ± SEM).
Histological assay of tissues
Macroscopic test of all organs including liver, spleen, kidney, centre, lung and brain tissue did not show any changes 24 h, 7, and 14 days after the administration of AuNPd (ane mg/kg), as compared to organs of control mice. No evidence of atrophy, hyperplasia, necrosis or inflammation was observed. To further explore for abnormalities, histological exam of tissues was performed using hematoxylin-eosin staining. Liver test did not evidence any negative effects in animals injected with AuNPd compared to control grouping. The microscopic parenchymal architecture evidenced normal hepatocytes without any signs of steatosis, inflammation nor fibrosis. Similarly, microscopic observation of kidney, spleen and centre did non reveal whatsoever sign of inflammation or fibrosis (Fig. v).
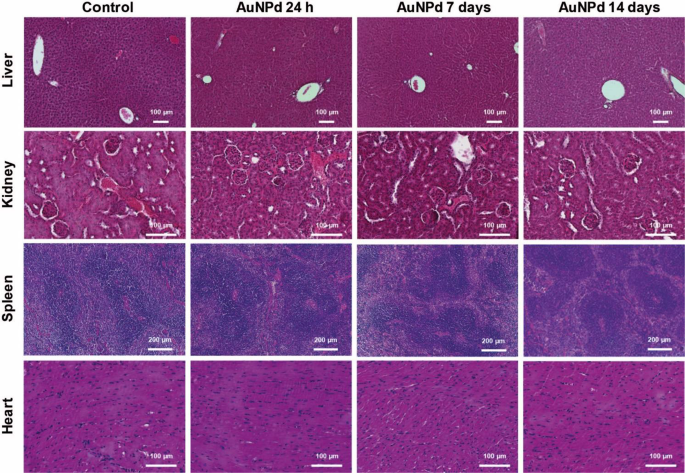
Histological department of mice liver, kidney, spleen and heart, 24 h, 7 and 14 days later intravenous assistants of AuNPd (i mg/kg) compared to the control group administered with vehicle alone. All sections were stained with hematoxylin-eosin.
Uptake and subcellular localization of AuNPd in hepatic and splenic tissues
Biodistribution information accept shown that AuNPd preferentially accumulated in liver and spleen. Therefore, we decided to assess subcellular localization of AuNPd in these organs past TEM at 24 h, 7 and 14 days after injection. A control group administered with vehicle alone was used. While gold is a dense-electron material, nanoparticles were easily detected on organ slides. AuNPd were observed in liver and spleen in all animals that receive AuNPd injection, and representative images are shown in Fig. half dozen. AuNPd were present within phagocytosing cells of both liver (Kupffer cells) and spleen (macrophages). No AuNPd was detected in other liver cells like hepatocytes or endothelial cells nor in lymphocytes and red blood cells in spleen. Although some AuNPd were found isolated in Kuppfer cells and macrophages (data not shown), AuNPd preferentially agglomerated in cluster (containing more than x particles). Isolated AuNPd or AuNPd clusters were localized in endolysosomal compartments. No AuNPd were detected in cytosol, nucleus or in other cytoplasmic organelles. No differences in terms of AuNPd quantity and subcellular localization were observed between 24 h, vii and xiv days post-injection. Kupffer cells and macrophages of control animals did not contain any AuNPd. In addition, no morphological changes such every bit chromatin condensation or mitochondrial morphology were detected in hepatic and splenic tissues of AuNPd injected animals compared to control mice. All together, our results prove that AuNPd accumulate in endolysosomes without inducing cellular toxicity (Fig. 6).
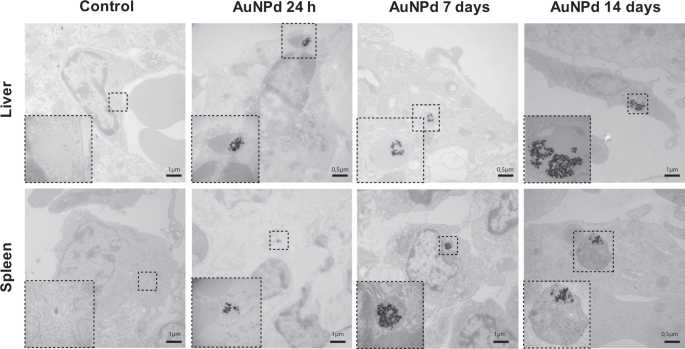
Representative TEM micrographs of Kupffer cells in liver and macrophages in spleen of mice 24 h, seven and 14 days after intravenous injection of AuNPd (1 mg/kg) compared to the control group administered with vehicle alone. Magnified views of the foursquare area show endolysosomal compartment containing clustered AuNPd in treated animals.
Biochemical and inflammation parameters subsequently AuNPd injection
Finally, nosotros investigated whether the handling with AuNPd could modify the levels of biochemical tissue harm or inflammatory markers in plasma. To decide if AuNPd induced liver toxicity, nosotros measured plasma ASAT (Aspartate Aminotransferase) and ALAT (Alanine Aminotransferase). An increase of these enzymes is considered as a fundamental marking of hepatocellular injury. ALAT and ASAT levels remained normal in AuNPd treated animals for 14 days every bit compared to the control grouping with a mean value around 183 U/Fifty and 35 U/Fifty for ASAT and ALAT, respectively (Fig. 7A). These results, combined with histological assay, point that even if AuNPd accrue in liver, they do not alter hepatic functions. Kidney role was assessed by measuring creatinine plasma level. We did not observe whatever significant divergence between the AuNPd treated groups sacrificed at 24 h and 14 days and the control group. However, the group sacrificed 7 days afterward AuNPd injection demonstrated a slight but statistically meaning increase in creatinine level every bit compared to control group from 10.92 ± 0.51 to 13.02 ± 0.61 µmol/50 (p = 0.026) (Fig. 7B). Importantly, these values remain under the toxicity threshold and are consistent with the literature (fifteen–30 µmol/Fifty)55,56,57. Moreover, the increase in creatinine was transitory and go back to normal value 1 week later, suggesting the absence of kidney dysfunction. Combined together, these results requite evidence that AuNPd accumulation in liver and their small amount in kidney practise not affect organ functionality.
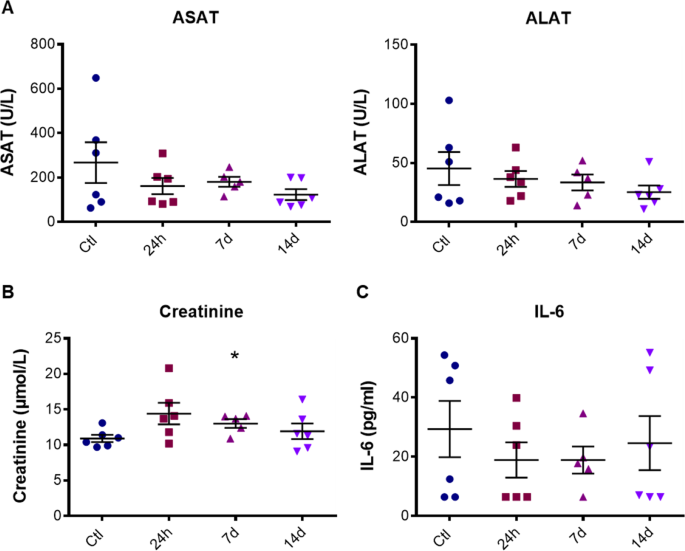
ALAT and ASAT values (A), creatinine level (B) and IL-six concentration (C) in mouse plasma 24 h, 7 or 14 days after AuNPd injection (1 mg/kg) or 14 days after vehicle injection for the command group. Data represent the hateful ± SEM of 6 mice and statistical significance was determined by Student's t-test (*p < 0.05).
The potential inflammatory outcome of AuNPd was adamant by plasma level of interleukin-vi (IL-6), a well-recognized mediator of inflammation. In command group, the boilerplate level of IL-half dozen was around 30 pg/ml as described in literature56. Comparing to the control group, IL-six level was not significantly modified at 24 h, seven days and 14 days postal service-treatment (Fig. 7C). These results confirmed that a unique intravenous injection of 1 mg/kg AuNPd was safe and did not induce astute and chronic toxicity.
Discussion
This study focused on the in vivo pharmacokinetics, biodistribution and toxicity of AuNP synthetized by ablation laser in dextran solution, following a bolus intravenous administration to subcutaneous tumor grafted mice. The healthy behaviour of animals, as well as the absence of acute and chronic toxicity on kidney, spleen and liver, confirm the condom of AuNPd previously described in vitro 54. Pharmacokinetic data have shown that AuNPd were promptly cleared from the bloodstream and accumulated preferentially in liver and spleen. Pocket-sized quantities of aureate were too present in kidney, lung, middle and brain. Our results are consistent with the pharmacokinetic contour described in the literature for AuNPd non coated with PEG58. Indeed, liver has largely been described equally a primal organ for nanoparticles accumulation (predominantly inside Kupffer cells) after the intravenous administration59. Form, size, charges and density of Au-NP modulate tissue distributionsixty. In a study on tissue distribution of spherical AuNPd in rat, Jong et al., show that large AuNPd (diameter betwixt l and 200 nm) well-nigh solely distributed in the blood, liver and spleen whereas smaller AuNPd (x nm diameter) were largely distributed61. In our report, gold content in liver and spleen did not decreased over time, suggesting a poor clearing efficacy through the bile ducts. A very low amount of AuNPd (0.23% of the injected dose) was found in kidney vii days after administration. The decrease in gilded content in kidney measured at solar day 14 every bit compared with day 7 may exist explained by the clearance in urine (≤0.03 ng/mL) of the smaller AuNPd (<8 nm) due to the physical size of glomerular filtration62. Because AuNPd predominantly accumulated in the liver, spleen and kidney, measurements of biochemical parameters and histopathological analysis were performed to deeply investigate their safe/toxicity risk for principal organs. Since plasma ALAT, ASAT activities and creatinine level remained virtually unchanged and comparable to the parameters of the control mice group, 1 can conclude that the accumulation of AuNPd did not provoke any hepatic or renal toxicity. Furthermore, we detect no sign of histological impairment such as fibrosis or inflammation in tissue. Lastly, the absence of chronic inflammation was supported by plasma IL-6 level that remains normal for 14 days after the AuNPd administration.
Since non-specific uptake of nanoparticles can occur in populations of phagocytic cells and dense capillary beds present in non-target organs, we likewise quantified gold in cardiac and pulmonary tissues. Very depression concentrations of gold were observed in these organs, thus confirming a proficient tolerability of AuNPd. In accordance with previous data26, less than 1% of gilt was detectable in brain. The smaller size of NP may have crossed the blood brain barrier (BBB) through the 20 nm space betwixt astrocytic end-anxiety basement membrane and capillary endothelium63. Alternatively, adsorption of apolipoproteins on golden NPd observed in our previous report54 could have further facilitated encephalon transport through interaction of apolipoprotein with the scavenger receptor class B type located at the BBB64. Moreover, surfactants coated at the surface of nanoparticles may meliorate anticancer drug accumulation in brain tumors65. Importantly, no cognitive or compartmental abnormal sign was detected in living animals, suggesting that AuNPd can be useful for brain tumor treatment as drug delivery vector or photothermotherapy after optimizing brain uptake. Work is in progress in this direction to improve brain targeting by BBB opening using ultrasound. Focused ultrasound mediated-BBB opening is a non-invasive technique where ultrasounds are used to temporarily open the BBB in highly targeted areas of the brain66.
Accumulation of nanoparticles in tumor by a passive targeting way is in competition with the internalization and clearance from blood by phagocytic cells and macrophages67. In addition, nanoparticles are supposed to be small enough to reach the tumor through transvascular pores and fenestrations for efficient EPR effect. Here, gilt was not detectable in tumor, which is consistent with the brusk blood one-half-life. Indeed, previous reports take shown that an increase in plasma half-life resulted in greater accumulation in the tumor67. All the same, the about efficient passive tumor targeting with AuNP remain lower than 8% of the injected dose per gram of tissue68. Moreover, Jinbin Liu et al., described a GS-AuNP with rapid clearance in normal tissue, long retention in tumors, and high tumor targeting specificity simply less than 5% AuNP per gram of tissue in tumor was observed at 12 h postal service injection69. In order to increase AuNPd aggregating in tumor, the quantity of dextran at the surface of AuNPd should be increased or another surfactant such as polyethylene glycol (PEG) could exist used. PEG has been previously shown to protect NP against opsonization and induce an increase in claret circulation time and enhanced tumor accumulationseventy. Alternatively, less negative zeta potential could increase the claret apportionment time. Indeed, Arvizo et al. has plant that neutral and zwitterionic AuNPd had a longer blood apportionment time while negatively and positively charged AuNP had a relatively brusque blood half-lives71.
In general, the level of toxicity we observed appears to be very low compared to previous studies on the use of chemically-prepared NP23,24,25. Information technology should be noted that the observed residue accumulation of aureate in the organism was mentioned in virtually all previous studies23,24,71. Withal, our data testify that the accumulation of laser-synthesized AuNP does not crusade any astute or chronic toxicity, as it tin can take place with alternative Au-based counterparts or many other nanomaterials. As an case, residual accumulation of silica (SiO2) nanoparticles in liver under similar atmospheric condition induced mononuclear inflammatory infiltrate at the portal surface area and hepatocyte necrosis at the portal triads seven days later on injection72, equally well the top in ASAT level and a larger number of sinusoidal Kupffer cells 48 h after intravenous assistants of rats55,72,73. We suppose that Au nanoparticles could be completely eliminated from the organism if their size is within renal glomerular filtration range (<eight nm)62. It is of import that laser-ablation technology makes possible the fabrication of both bare and polymer-coated AuNP within this range46,47,48. Interestingly, nosotros recently carried our like study of safety and biodistribution of laser-synthesized Si nanoparticles56,74, which nowadays another promising material for imaging and therapy applications in biomedicine75, just in contrast to Au nanostructures76 Si nanoparticles are biodegradable56,77,78. Our experiments too evidenced preferable accumulation of bare (uncoated) Si NP in liver and spleen under their intravenous administration, but such an accumulation did not crusade any toxicity effects56,78. In add-on, in contrast to golden nanostructures Si NP were apace biodegraded in the organism and completely eliminated iv–6 days following their injection56.
Conclusions
For the first time nosotros studied safety, pharmacokinetics and biodistribution of light amplification by stimulated emission of radiation-synthesized dextran-coated AuNP under intravenous administration in pocket-sized animal model. Nosotros found that AuNP were by and large accumulated in liver and spleen, but did not event in any hepatic or renal toxicity, as was indicated by stability of biochemical parameters and histological examination of tissues. The condom of AuNPd was confirmed by healthy behavior of animals and the absence of acute and chronic toxicities in key organs. In general, despite residual accumulation in tissues, laser-synthesized nanoparticles present a safe and very promising object for biomedical applications and can exist used u.s.a. drug carriers, contrast agents or sensitizers of therapies.
Methods
Synthesis of Au nanoparticles
For the fabrication of dextran-coated Au nanoparticles, nosotros used a Yb:KGW laser (Amplitude Systems, 1025 nm, 480 fs, 100–200 μJ per pulse, i–100 kHz). The laser axle was focused onto the surface of s gold target (99.99%, GoodFellow, Lille, France) placed on the glass vessel bottom filled with seven ml of deionized h2o containing ane mg/mL of dextran (MW ~40,000, Sigma-Aldrich). To avert the formation of craters leading to the subtract of ablation efficiency, the target was moved at a speed of 0.v mm/south during the experiment. To measure out concentration of formed nanoparticles, we measured weight of the ablated target before and after laser ablation process.
Characterization of nanoparticles
We used a high-resolution manual electron microscopy system (JEOL JEM 3010) to assess structural characteristics of formed nanoparticles. To prepare samples, we dropped a droplet (about v μL) of AuNP solution onto a carbon-coated TEM copper grid and dried it at ambient conditions. To examine extinction spectra, we used a UV–VIS spectrophotometer (UV–2600, Shimadzu).
In vivo study pattern
All experimental protocols and animal analyses were conducted in accordance with the guidelines of the French Regime and the Regional Commission for Ethics on Fauna Experiments (authority number 0100903). The experimental procedure was approved by the Committee for Ethics on Animal Experiments of the Establish of NeuroPhysiopathology. For the biodistribution study, 24 athymic nude female mice (Athymic Nude-Foxn1nu) (Harlan, France) aged vi weeks were randomly divided in 4 groups. Mice were housed in cages, located in a well-ventilated, temperature-controlled room 21 ± 2 °C with relative humidity ranging from 40% to 60%, and a light/night menses of 12 h, with free admission to water and food. On twenty-four hours 0, 2.5 × 10six U87-MG human glioblastoma cells were administered subcutaneously on the left flank of all mice. Tumor growth and trunk weight were monitored twice a week. On day 14, when tumor measured approximately 100 mm3, iii groups of 6 mice were intravenously administered in tail vein with a single dose of 1 mg/kg dextran-coated gilded nanoparticles (AuNPd) diluted in phosphate buffer saline (PBS), corresponding to the maximal volume that tin can exist administered intravenously. Command mice were injected through the tail vein only with PBS. Afterward AuNPd administration, mice trunk weight and behaviour were monitored to detect a possible toxic event of NPs. Animals were sacrificed at different times afterward AuNPd injection: 24 h, 7 days and 14 days. 20-4 hours before cede, three mice per grouping were housed individually in metabolic cages to recover urine. Mice were then anesthetized with a solution of ketamine (0.75 mg/kg trunk weight) and xylazine (0.x mg/kg trunk weight), and exsanguinated by cannulating the posterior aorta. The liver, spleen, kidneys, lungs, heart, brain, tumor were removed and candy for histological and electron microscopy analysis as described below. Samples dedicated to gold decision were frozen and stored at −20 °C before assay. The organs of 3 mice per group were used for gilded determination and histological analysis. The organs of the 3 other mice were used for electron microscopy analysis.
For the pharmacokinetic study, 35 athymic nude female mice (Athymic Nude-Foxn1nu) (Harlan, France) aged six weeks were used and randomly divided in 7 groups. Mice were administered intravenously with the maximal dose of 1 mg/kg AuNPd. Animals were sacrificed at different time points: v min, 15 min, 30 min, 45 min, 60 min, 4 h and 24 h after AuNPd injection and blood samples (800 µL) were collected past intra-cardiac puncture. Samples dedicated to gold decision were frozen and stored at −20 °C earlier analysis.
Gold determination content
Biological samples including liver, spleen, lung, kidney, heart, brain, tumor, were cut in small pieces and mineralized with nitric acrid (3 M) / muriatic acid (1 M) and incubated at 100 °C during eight h. Liquid samples including whole claret and urine were mineralized by improver of i ml of acrid solution. Mineralized pellets were and then diluted in deionized water and analyzed by ICP-MS using a Thermo Series Two ICP-MS apparatus (Thermo-Electron, Les Ulis, France) to make up one's mind Au concentration. Standard calibration curve was performed with a solution of ionic gold and a solution of AuNP. (Quantification threshold was fixed at 0.01 ng/mg for tissues and 0.006 ng/µL for urine and blood).
Biochemical analysis
Blood samples were collected by intra-cardiac puncture; plasmas were prepared by two successive centrifugations at 382 thousand (2000 rpm) for 20 minutes. Samples were stored at −20 °C until assay by Institut clinique de la souris; Illkirch-Graffenstaden. ALAT (Alanine AminoTransferase), ASAT (Aspartate AminoTransferase) and creatinine plasmatic levels were quantified using AU400 Chemical science Analyzer, Beckman Coulter. Interleukin-6 plasmatic level was quantified by immunoassay using Mouse Cytokine/Chemokine Magnetic Bead Panel (IL-6) (Millipore, MCYTOMAG-70k).
Histological analysis
Organs were carefully collected, fixed and conserved in formalin solution before methane series-embedding. Three-µm-thick alkane series sections of different organs were and then processed manually. Slides were deparaffinized in three successive baths of xylene (Hydroclear) for 10 min so rehydrated twice with 100% ethanol. Subsequently rising in tap h2o for x min, slides were stained with Gründwald Hematoxylin for thirty s. After washing, slides were stained with eosin for 15 due south and quickly rinsed in tap water. Three baths of 100% ethanol were realized, and slides were stained in alcoholic safran solution for 30 seconds. Slides were so cleared in xylene three times and mounted with Eukitt medium. The stained slides were scanned on a whole slide scanner (Nanozoomer 2.0-HT, Hamamatsu, Japan) at × 40 magnification, obtained images were opened using NP-Viewer software.
Transmission electron microscopy studies of biological samples
For TEM, samples were candy as followed. Iii mice per grouping were perfused with 2.0% paraformaldehyde, 2.5% EM course glutaraldehyde in PBS (pH 7.4). Organs were then carefully collected and cut into 1 mm3 cubes in perfusion solution under binocular loupe. Samples were then fixed in ii.0% paraformaldehyde, 2.5% EM grade glutaraldehyde, 0.one% tannic acid in 0.one One thousand sodium cacodylate buffer (pH 7.4). After fixation, samples were washed twice in 0.i M sucrose 0. ane Chiliad sodium cacodylate buffer (pH 7.4) and post-fixed in ii% osmium tetroxide in 0.1 Yard sodium cacodylate buffer (pH vii.four) for ane h. Samples were washed once with 0.1 K sucrose 0.i One thousand sodium cacodylate buffer and thrice in deionized water to eliminate excess of osmium. Sample dehydration started with 15 min 30% ethanol bath and a second in l% ethanol. At this stride a first tissue contrast was realized in 1% uranyl acetate in 70% ethanol at 4 °C on night. Sample dehydration continued with 40 min successive baths of 70, 90, 95 and 100% ethanol and finished with a 100% acetone bath. Samples in solvent were progressively infiltrated with increasing concentration of Epon in an Epon/aceton mix. In one case in 100% Epon, sample were disposed in Epon into flat embedding molds and put in oven at threescore °C for 48 h for polymerization. Ultrathin sections of 80 nm were cut with a diatom diamond knife on a LBK ultramicrotome Leica UCT and placed on coated square mesh copper grids. Sections were so counterstained with uranyl acetate and Reynold's lead citrate and viewed with a FEI Tecnai G2 electron microscope. Digital images were acquired with a Veleta Camera (Olympus).
Statistics
Data were analyzed for statistical significance using GraphPad Prism software.
References
-
Cunningham, D. A. H., Vogel, W., Kageyama, H., Tsubota, S. & Haruta, Thousand. The Relationship betwixt the Construction and Activity of Nanometer Size Gold When Supported on Mg(OH)2. J. Catal. 177, 1–x (1998).
-
Haruta, M. Aureate rush. Nature 437, 1098–1099 (2005).
-
Blizanac, B. B. et al. Anion Adsorption, CO Oxidation, and Oxygen Reduction Reaction on a Au(100) Surface: The pH Effect. J. Phys. Chem. B 108, 625–634 (2004).
-
Hebié, S. et al. Advanced Electrocatalysts on the Basis of Bare Au Nanomaterials for Biofuel Cell Applications. ACS Catal. five, 6489–6496 (2015).
-
Jain, P. K., Lee, 1000. S., El-Sayed, I. H. & El-Sayed, Chiliad. A. Calculated absorption and scattering properties of gilt nanoparticles of different size, shape, and composition: applications in biological imaging and biomedicine. J. Phys. Chem. B 110, 7238–7248 (2006).
-
Nie, S. Probing unmarried molecules and single nanoparticles past surface-enhanced Raman handful. Science 275, 1102–1106 (1997).
-
Lal, S., Link, S. & Halas, N. J. Nano-optics from sensing to waveguiding. Nature Photon. 1, 641–648 (2007).
-
Kabashin, A. V. et al. Plasmonics nanorod metamaterials for biosensing. Nature Mater. 8, 867–871 (2009).
-
Paciotti, G. F. et al. Colloidal gold: A novel nanoparticle vector for tumor directed drug delivery. Drug Delivery 11, 169–183 (2004).
-
Connor, E. E., Mwamuka, J., Gole, A., Murphy, C. J. & Wyatt, M. D. Gold nanoparticles are taken up past human cells but exercise not cause astute cytotoxicity. Pocket-sized 1, 325–327 (2005).
-
Dreaden, E. C., Alkilany, A. M., Huang, X., Irish potato, C. J. & El-Sayed, G. A. The golden historic period: gilt nanoparticles for biomedicine. Chem. Soc. Rev. 41, 2740–2779 (2012).
-
Hirsch, L. R. et al. Nanoshell-mediated near-infrared thermal therapy of tumors under magnetic resonance guidance. Proc. Natl. Acad. Sci. 100, 13549–13554 (2003).
-
Loo, C., Lowery, A., Halas, N., Westward, J. & Drezek, R. Immunotargeted nanoshells for integrated cancer imaging and therapy. Nano Lett. v, 709–711 (2005).
-
Huang, X., El-Sayed, I. H., Qian, West. & El-Sayed, M. A. Cancer cell imaging and photothermal therapy in the near-infrared region by using gold nanorods. J. Am. Chem. Soc. 128, 2115–2120 (2006).
-
Sokolov, K. et al. Real-fourth dimension vital optical imaging of precancer using anti-epidermal growth factor receptor antibodies conjugated to gold nanoparticles. Cancer Res. 63, 1999–2004 (2003).
-
Gobin, A. M. et al. Near-infrared resonant nanoshells for combined optical imaging and photothermal cancer therapy. Nano Lett. vii, 1929–1934 (2007).
-
Wang, Y. et al. Photoacoustic tomography of a nanoshell contrast amanuensis in the in vivo rat brain. Nano Lett. 4, 1689–1692 (2004).
-
Cole, L. E., Ross, R. D., Tilley, J. Thousand., Vargo-Gogola, T. & Roeder, R. Thousand. Gold nanoparticles equally dissimilarity agents in x-ray imaging and computed tomography. Nanomedicine 10, 321–41 (2015).
-
Ahn, Southward., Jung, S. Y. & Lee, S. J. Golden Nanoparticle Contrast Agents in Advanced Ten-ray Imaging Technologies. Molecules eighteen, 5858–5890 (2013).
-
Jain, S., Hirst, D. Chiliad. & O'Sullivan, J. Thou. Gold nanoparticles as novel agents for cancer therapy. British Journal of Radiology 85, 101–113 (2012).
-
Haume, G. et al. Aureate nanoparticles for cancer radiotherapy: a review, Cancer. Nano 7, 8 (2016).
-
Ngwa, W. et al. Targeted radiotherapy with gold nanoparticles: current status and future perspectives. Nanomedicine 9, 1063–1082 (2014).
-
Sabella, S., Galeone, A., Vecchio, G., Cingolani, R. & Pompa, P. P. AuNPs are toxic in vitro and in vivo: A review. J. Nanosci. Lett. 1, 145–165 (2011).
-
Yah, C. South. The toxicity of gold nanoparticles in relation to their physicochemical properties. Biomed. Res. 24, 400–413 (2013).
-
Fratoddi, I., Venditti, I., Cametti, C. & Russo, M. 5. How toxic are golden nanoparticles? The State-of-the-Art. Nano Res. eight, 1771–1799 (2015).
-
Khlebtsov, N. & Dykman, L. Biodistribution and toxicity of engineered gilded nanoparticles: a review of in vitro and in vivo studies. Chem. Soc. Rev. 40, 1647–1671 (2011).
-
Brust, 1000., Walker, M., Bethell, D., Schiffrin, D. J. & Whyman, R. Synthesis of thiol-derivatised gold nanoparticles in a ii-phase Liquid–Liquid system. J. Chem. Soc., Chem. Commun. 0, 801 (1994).
-
Kimling, J. et al. Turkevich Method for Gilt Nanoparticle Synthesis Revisited. J. Phys. Chem. B 110, 15700 (2006).
-
Gole, A. & White potato, C. Seed-Mediated Synthesis of Gilt Nanorods: Function of the Size and Nature of the Seed. J. Chem. Mater. sixteen, 3633 (2004).
-
Lohse, Southward. East. & Murphy, C. J. Polyelectrolyte-Coated Gold Nanorods: Synthesis, Label and Immobilization. Chem. Mater. 25, 1250 (2013).
-
Tedesco, Southward., Doyle, H., Redmond, 1000. & Sheehan, D. Gold nanoparticles and oxidative stress in mytilus edulis. Marine Environ. Res. 66, 131–133 (2008).
-
Kong, B., Seog, J. H., Graham, L. K. & Lee, Southward. B. Experimental considerations on the cytotoxicity of nanoparticles. Nanomedicine 6, 929–941 (2011).
-
Gerber, A., Bundschuh, Grand., Klingelhofer, D. & Groneberg, D. A. Gilt nanoparticles: Recent aspects for human toxicology. J. Occup. Med. Toxic. 8, 32 (2013).
-
Alkilany, A. M. et al. Cellular uptake and cytotoxicity of gilded nanorods: Molecular origin of cytotoxicity and surface furnishings. Modest 5, 701–708 (2009).
-
Railsback, J. G. et al. Weakly charged cationic nanoparticles induce Deoxyribonucleic acid angle and strand separation. Adv. Mater. 24, 4261–4265 (2012).
-
Paillusson, F., Dahirel, V., Jardat, Thou., Victor, J. M. & Barbo, Thou. Effective interaction betwixt charged nanoparticles and DNA. Phys. Chem. Chem. Phys. xiii, 12603–12613 (2011).
-
Rayavarapu, R. G. et al. In vitro toxicity studies of polymer-coated gold nanorods. Nanotechnology. 21, 145101 (2010).
-
Fojtik, A. & Henglein, A. Laser Ablation of Films and Suspended Particles in a Solvent - Germination of Cluster and Colloid Solutions. Ber Bunsen. Phys Chem 97, 252–254 (1993).
-
Neddersen, J., Chumanov, G. & Cotton, T. M. Laser-Ablation of Metals - a New Method for Preparing SERS Active Colloids. Appl Spectroscopy 47, 1959–1964 (1993).
-
Kabashin, A. 5. & Meunier, M. Synthesis of colloidal nanoparticles during femtosecond laser ablation of gold in water. J. Appl. Phys. 94, 7941 (2003).
-
Kabashin, A. V. & Meunier, M. Femtosecond light amplification by stimulated emission of radiation ablation in aqueous solutions: a novel method to synthesize not-toxic metallic colloids with controllable size. J. Phys. Conf. Ser. 59, 354–359 (2007).
-
Kabashin, A. V. et al. Nanofabrication with Pulsed Lasers. Nanoscale Res. Lett. v, 454–463 (2010).
-
Sylvestre, J. P., Kabashin, A. V., Sacher, E. & Meunier, Grand. Femtosecond laser ablation of gold in water: Influence of the laser-produced plasma on the nanoparticle size distribution. Appl. Phys. A Mater. Sci. Procedure. lxxx, 753–758 (2005).
-
Besner, Due south., Kabashin, A. V. & Meunier, One thousand. Fragmentation of colloidal nanoparticles by femtosecond laser-induced supercontinuum generation. Appl. Phys. Lett. 89, 233122 (2006).
-
Maximova, G., Aristov, A., Sentis, M. & Kabashin, A. V. Size-controllable synthesis of bare gold nanoparticles by femtosecond light amplification by stimulated emission of radiation fragmentation in h2o. Nanotechnology 26, 065601 (2015).
-
Sylvestre, J. P. et al. Surface chemistry of gold nanoparticles produced past laser ablation in aqueous media. J. Phys. Chem. B 108, 16864–16869 (2004).
-
Kabashin, A. V., Meunier, M., Kingston, C. & Luong, J. H. T. Fabrication and label of gold nanoparticles by femtosecond laser ablation in aqueous solution of cyclodextrins. J. Phys. Chem. B. 107, 4527–4531 (2003).
-
Besner, S., Kabashin, A. V., Meunier, 1000. & Winnik, F. Thousand. Synthesis of size-tunable polymer-protected gold nanoparticles past femtosecond laser-based ablation and seed growth. J. Phys. Chem. C 113, 9526–9531 (2009).
-
Petersen, Southward. et al. Penetratin-conjugated gilded nanoparticles: blueprint of cell-penetrating nanomarkers by femtosecond laser ablation. J. Phys. Chem. C 115, 5152–5159 (2011).
-
Petersen, Due south. & Barcikowski, S. In situ bioconjugation: single step approach to tailored nanoparticle-bioconjugates by ultrashort pulsed laser ablation. Adv Funct Mater. xix, 1167–1172 (2009).
-
Sobhan, M. A. et al. Non-specific internalization of laser ablated pure gold nanoparticles in pancreatic tumour cell. Colloid Surface B. 92, 190–195 (2012).
-
Taylor, U. et al. Nonendosomal cellular uptake of ligand-gratis, positively charged gold nanoparticles. Cytom Role A. 77, 439–446 (2010).
-
Salmaso, S. et al. Prison cell upward-accept control of gilt nanoparticles functionalized with a thermoresponsive polymer. J Mater Chem. 19, 1608–1615 (2009).
-
Correard, F. et al. Gold nanoparticles prepared past laser ablation in aqueous biocompatible solutions: assessment of rubber and biological identity for nanomedicine applications. Int. J. Nanomedicine nine, 5415–30 (2014).
-
Chen, Q., Xue, Y. & Lord's day, J. Kupffer jail cell-mediated hepatic injury induced by silica nanoparticles in vitro and in vivo. Int. J. Nanomedicine. viii, 1129–1140 (2013).
-
Baati, T. et al. Ultrapure laser-synthesized Si-based nanomaterials for biomedical applications: in vivo assessment of safety and biodistribution. Sci. Rep. half dozen, 25400 (2016).
-
Yuen, P. S. T. et al. A simplified method for HPLC determination of creatinine in mouse serum. Am. J. Physiol. Physiol. 286, F1116–F1119 (2004).
-
Wang, J. et al. Size- and surface chemistry-dependent pharmacokinetics and tumor aggregating of engineered golden nanoparticles later on intravenous administration. Metallomics seven, 516–524 (2015).
-
Sadauskas, E. et al. Kupffer cells are cardinal in the removal of nanoparticles from the organism. Function. Fibre Toxicol. 4, 10 (2007).
-
Cho, W.-S. et al. Size-dependent tissue kinetics of PEG-coated gold nanoparticles. Toxicol. Appl. Pharmacol. 245, 116–123 (2010).
-
Jong, Due west. H. et al. Particle size-dependent organ distribution of gold nanoparticles afterward intravenous administration. Biomaterials 29, 1912–1919 (2008).
-
Zhang, J. et al. Quantitative Biokinetics and Systemic Translocation of Diverse Gold Nanostructures Are Highly Dependent on Their Size and Shape. J. Nanosci. Nanotechnol. 14, 4124–4138 (2014).
-
Hawkins, B. T. & Davis, T. P. The Blood-Brain Barrier/Neurovascular Unit in Wellness and Affliction. Pharmacol. Rev. 57, 173–185 (2005).
-
Takeuchi, I., Nobata, S., Oiri, N., Tomoda, 1000. & Makino, K. Biodistribution and excretion of colloidal gilded nanoparticles after intravenous injection: Effects of particle size. Biomed. Mater. Eng. 28, 315–323 (2017).
-
Petri, B. et al. Chemotherapy of brain tumour using doxorubicin bound to surfactant-coated poly(butyl cyanoacrylate) nanoparticles: Revisiting the role of surfactants. J. Command. Release 117, 51–58 (2007).
-
Marty, B. et al. Dynamic study of claret-encephalon bulwark closure later its disruption using ultrasound: A quantitative analysis. J. Cereb. Blood Flow Metab. 32, 1948–1958 (2012).
-
Perrault, Due south. D., Walkey, C., Jennings, T., Fischer, H. C. & Chan, Due west. C. W. Mediating Tumor Targeting Efficiency of Nanoparticles Through Blueprint. Nano Lett. ix, 1909–1915 (2009).
-
Wang, Y. et al. Evaluating the Pharmacokinetics and In Vivo Cancer Targeting Capability of Au Nanocages past Positron Emission Tomography Imaging. ACS Nano 6, 5880–5888 (2012).
-
Liu, J. et al. Passive Tumor Targeting of Renal-Clearable Luminescent Gold Nanoparticles: Long Tumor Retentivity and Fast Normal Tissue Clearance. J. Am. Chem. Soc. 135, 4978–4981 (2013).
-
Zhang, One thousand. et al. Influence of anchoring ligands and particle size on the colloidal stability and in vivo biodistribution of polyethylene glycol-coated gold nanoparticles in tumor-xenografted mice. Biomaterials 30, 1928–1936 (2009).
-
Arvizo, R. R. et al. Modulating Pharmacokinetics, Tumor Uptake and Biodistribution past Engineered Nanoparticles. PLoS 1 six, e24374 (2011).
-
Xie, Thousand. et al. Biodistribution and toxicity of intravenously administered silica nanoparticles in mice. Arch. Toxicol. 84, 183–190 (2010).
-
Edmiston et al. In vivo induction of murine product by carcinoembryonic antigen. Cancer Res. 57, 4432–4436 (1997).
-
Al-Kattan et al. Ultrapure laser-synthesized Si nanoparticles with variable oxidation state for biomedical applications. J. Mater. Chem. B 4, 7852 (2016).
-
Kabashin, A. Five. & Timoshenko, V. Yu. What theranostic applications could ultrapure light amplification by stimulated emission of radiation-synthesized Si nanoparticles have in cancer? Nanomedicine 11, 2247–2250 (2016).
-
James, W. D., Hirsch, L. R., West, J. L., O'Neal, P. D. & Payne, J. D. Application of INAA to the build-up and clearance of gilt nanoshells in clinical studies in mice. J. Radioanal. Nucl. Chem. 271, 455–459 (2007).
-
Park, J. H. et al. Biodegradable luminescent porous silicon nanoparticles for in vivo applications. Nat. Mater. eight, 331–336 (2009).
-
Petriev, V. M. et al. Nuclear nanomedicine using Si nanoparticles as safe and effective carriers of 188Re radionuclide for cancer therapy. Sci. Rep. 9, 2017 (2019).
Acknowledgements
The authors thank Dr. Raphael Berges for technical assistance in creature experiments, the animal facility "Centre d'Exploration Fonctionnelle Scientifique" (CEFOS) agreement East thirteen-055-5, campus Timone Aix-Marseille University, the PICsL-FBI core electron microscopy facility (Aïcha Aouane, Fabrice Richard and Nicolas Brouilly, IBDM, AMU-Marseille UMR 7288), fellow member of the national infrastructure France-BioImaging supported by the French National Enquiry Agency (ANR-x-INBS-0004). The authors acknowledge the contribution from ITMO cancer AVIESAN (National Brotherhood for the Life Sciences & Health) within the framework of the Cancer Plan (GRAVITY Projection PC201613). A.P. and A.V.One thousand. acknowledge support from the MEPhI Academic Excellence Project (Contract No. 02.a03.21.0005). A.P. and A.V.K. also acknowledge of Russian Science Foundation (Project 19-72-30012) for the fabrication of samples of highly calibrated nanoparticles.
Author information
Affiliations
Contributions
D.B. and M.A.E and A.V.K. conceived and designed the enquiry. A.A.P., G.T., A.A.K., A.V.K. designed laser synthesis experiments, fabricated and characterized AuNP. A.50.B., F.C., D.B. and Chiliad.A.Due east. designed and performed cytotoxicity, jail cell uptake tests, and in vivo studies. A.L.B., F.C., F.Ch. did ICP-MS assays of gold. R.A. participated to histology experiments and information analysis. All authors analyzed the data. D.B. and A.5.Thousand. guided the project. A.Five.Chiliad. arranged the final version of the manuscript using data from co-authors. All authors have given approving to the final version of the manuscript. A.Fifty.B. and F.C. contributed as to this manuscript.
Respective authors
Ethics declarations
Competing Interests
The authors declare no competing interests.
Additional information
Publisher's note: Springer Nature remains neutral with regard to jurisdictional claims in published maps and institutional affiliations.
Rights and permissions
Open Access This article is licensed under a Artistic Commons Attribution 4.0 International License, which permits employ, sharing, accommodation, distribution and reproduction in any medium or format, as long equally you give appropriate credit to the original author(southward) and the source, provide a link to the Creative Commons license, and bespeak if changes were made. The images or other third party fabric in this article are included in the article's Artistic Commons license, unless indicated otherwise in a credit line to the material. If material is non included in the article's Creative Commons license and your intended employ is not permitted by statutory regulation or exceeds the permitted apply, you lot will need to obtain permission directly from the copyright holder. To view a copy of this license, visit http://creativecommons.org/licenses/by/4.0/.
Reprints and Permissions
About this article
Cite this article
Bailly, AL., Correard, F., Popov, A. et al. In vivo evaluation of safety, biodistribution and pharmacokinetics of laser-synthesized gold nanoparticles. Sci Rep 9, 12890 (2019). https://doi.org/10.1038/s41598-019-48748-three
-
Received:
-
Accustomed:
-
Published:
-
DOI : https://doi.org/10.1038/s41598-019-48748-3
Further reading
Comments
By submitting a annotate you lot concur to abide by our Terms and Community Guidelines. If you find something abusive or that does not comply with our terms or guidelines please flag it as inappropriate.
Source: https://www.nature.com/articles/s41598-019-48748-3?error=cookies_not_supported&code=903210d3-ed52-4240-969d-9013b63e960d
0 Response to "Railsback, J., & Corey B. (2003). Building Trust With Schools and Diverse Families."
Post a Comment